Introduction
Current understanding of the molecular events underlying muscle contraction is embodied in the sliding filament model of muscle contraction. The model is applicable to smooth, skeletal, cardiac, and other contractile activity, including mechanochemical events such as single cell locomotion and receptor endocytosis. Since the biochemistry of these activities are best understood for skeletal muscle, this discussion focus on skeletal muscle (noting, where appropriate, differences in the other muscle types). The biochemical characteristics that differentiate fast-reacting and slow-reacting cells in muscle tissue and the biochemical basis of some common pathophysiological states of muscle, including tetany, fatigue, and rigor mortis are reviewed as well.
Skeletal muscles comprise about 40% of the mass of the average human body and are formed of long multinucleate, cylindrical cells called muscle fibers.
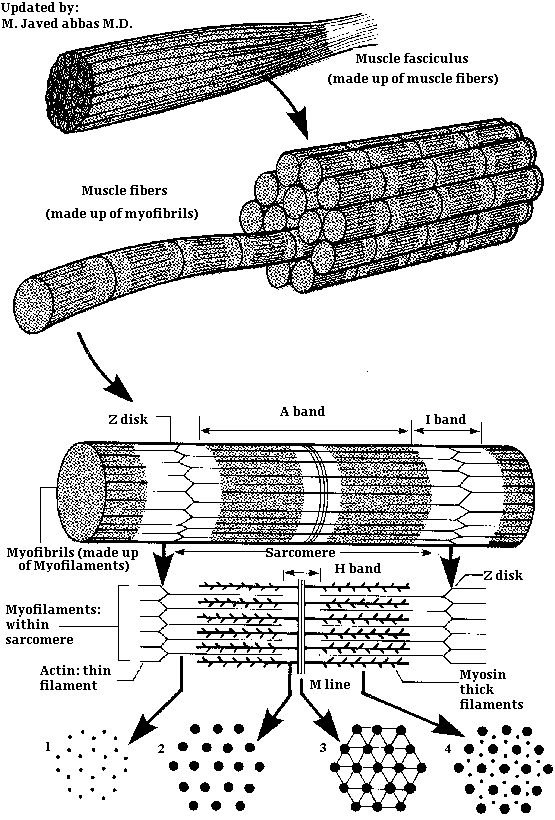 |
Diagrammatic breakdown of a typical muscle. Shows how actin thin filaments and myosin thick filaments are arranged to form the myofilaments of a sarcomere, continuing with the formation of myofibrils from many myofilaments. |
The plasma membrane of muscle fibers is known as the sarcolemma. Each muscle is made up of bundles of these fibers, or cells, embedded in a matrix of connective tissue known as the endomysium. The bundle of fibers with its endomysium is surrounded by a more fibrous connective tissue sheath known as the perimysium. The composite of the perimysium and its contents is known as a fasciculus. A complete muscle consists of numerous fasciculi surrounded by a thick outer layer of connective tissue known as the perimysial septa. The translation of contractile activity of individual muscle fibers to anatomical motion take place through this continuous system of connective tissues and sheaths, which ultimately meld into the tendons.
Within the sarcolemma is the sarcoplasm, containing all the usual subcellular elements plus long prominent myofibrils. Each myofibril is composed of bundles of filamentous contractile proteins, some extending from end to end in the cell. Myofibrils are the most conspicuous elements in skeletal myofibers making up about 60% of myofiber protein. A single myofibril is composed of many short structural units, known as sarcomeres, which are arranged end to end. The proteins at the junctions between sarcomeres form the Z line, and thus a sarcomere extends along a myofibril from one Z line to the next Z line. Sarcomeres are composed mostly of actin thin filaments and myosin thick filaments. Sarcomeres represent the minimal contractile unit of a muscle. It is the coordinated contraction and elongation of millions of sarcomeres in a muscle that gives rise to mechanical skeletal activity. The relationship between muscle proteins and muscles is summarized in the table below:
Organization of Contractile Proteins in Muscle |
Thick Filament |
Composed of hundreds of long, contractile myosin molecules arranged in a staggered side by side complex. |
Thin Filament |
Composed of a linear array of hundreds of globular, actin monomers in a double helical. arrangement. |
Sarcomere |
The unit of contractile activity composed mainly of actin and myosin and extending from Z line to Z line in a myofibril. |
Myofibril |
End to end arrays of identical sarcomeres. |
Myofiber |
A single multinucleate muscle cell containing all the usual cell organelles plus many myofibrils. |
Muscle |
Organized arrays of muscle fibers. |
back to the top
Organization of the Sarcomere
The organization of individual contractile proteins making up a sarcomere is a key feature of the sliding filament model. Each sarcomere is composed of hundreds of filamentous protein aggregates, each known as a myofilament. Two kinds of myofilaments are identifiable on the basis of their diameter and protein composition (see image above). Thick myofilaments are composed of several hundred molecules of a fibrous protein known as myosin. Thin myofilaments are composed of two helically interwound, linear polymers of a globular protein known as actin. Thin and thick filaments also contain accessory proteins, described below. Proteins of the Z line, including a-actinin, serve as an embedding matrix or anchor for one end of the thin filaments, which extend toward the center of sarcomeres on either side of the Z line. The Z line proteins often appear continuous across the width of a muscle fiber and seem to act to keep the myofibrils within a myofiber in register. The distal end of each thin filament is free in the sarcoplasm and is capped with a protein known as b-actinin.
Also depicted in the image above is a second disk-like protein aggregate: the M-line, which is centrally located in sarcomeres. Like Z line protein, the M line protein aggregate acts as an embedding matrix, in this case for the myosin thick filaments. Thick filaments extend from their point of attachment on both sides of the M line toward the two Z lines that define a sarcomere.
Within a sarcomere the thick and thin filaments interdigitate so that in cross section they are seen to form a hexagonal lattice, in which 6 thin filaments are arrayed around each thick filament. The thick filaments are also arranged hexagonally to each other. During contraction and relaxation the distance between the Z lines varies, decreasing with contraction and increasing with relaxation. The M line, with its attached thick filaments, remains centrally located in the sarcomere. The thin and thick filaments retain their extended linear structure except in extreme situations. Changes in sarcomere length are caused by the thin filaments being pulled along the thick filaments in the direction of the M line.
back to the top
Proteins of the Myofilaments
The biochemical basis of muscle activity is related to the enzymatic and physical properties of actin, myosin, and the accessory proteins that constitute the thin and thick filaments. The following discussion summarizes the key protein components of the myofilaments and their ATP-dependent interactions, which produce contractile activity.
The proteins of the thin and thick filaments can be separated into actin, myosin, and 6 accessory proteins. The accessory proteins are a-actinin, b-actinin, tropomyosin, troponin, C protein, and M line protein.
Solubilized myosin molecules are long thin (fibrous) proteins with a molecular weight of about 500,000 daltons.
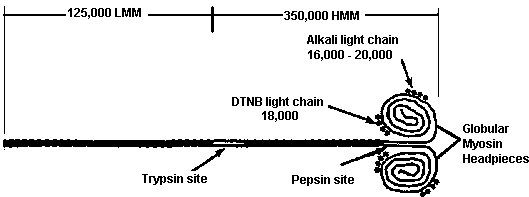 |
Diagram of a myosin molecule showing the intertwined a-helices of the fibrous portion of the heavy chains. The globular head region of the heavy chains associates with the alkali and DTNB light chains (so named for the methods used for their extraction). |
Each molecule is made up of 6 subunits, 2 very large, heavy chains (HC), and 4 smaller, light chains (LC). In a given muscle fiber the 2 large subunits are identical, although there are different HC isoforms in different types of muscle fibers. Heavy chains contain a long linear C-terminal a-helical domain (1,300 amino acids) and a prominent globular N-terminal domain of about 800 amino acids. The two HC, a-helical domains are helically interwound, giving the molecules a long, rigid superhelical structure with 2 globular headpieces. A complete myosin molecule also contains 4 relatively small proteins which are associated with the globular headpieces. These small proteins, of molecular weight 16,000--24,000 daltons, are known as alkali light chains (LC1 or LC3) and DTNB light chains (LC2). Each myosin molecule contains 2 subunits of LC2, 1 associated with each HC globular domain. Each of the globular domains also contains a subunit of either LC1 or LC3, with the proportions of LC1 and LC3 in the myosin molecules varying in myosins from cardiac, skeletal, embryonic, and smooth muscle. All light chains bind Ca2+ with high affinity, are phosphorylated by myosin light chain kinase (MLCK), and generally serve in the regulation of myosin's ATPase activity and its assembly into thick filaments
Several functionally important landmarks exist on the myosin molecule. Near the midpoint of the long linear superhelical region is a site defined by its ready susceptibility to proteolytic trypsin digestion. Trypsin cleaves myosin into 2 portions: 1 containing both globular headpieces and some superhelical region, and the other consisting of the remaining superhelical portion of the carboxy terminus. The portion containing the headpiece is known as heavy meromyosin (HMM; molecular weight 350,000). The C-terminal fragment is known as light meromyosin (LMM; molecular weight 125,000).
The significance of the trypsin site is that its susceptibility to protease action is thought to reflect an interruption in the otherwise rigid superhelix, allowing this site to act as one of a hinge point involved in converting the chemical energy of ATP into the mechanical events of contraction and relaxation. A second proteolytic landmark susceptible to papain has also been considered a hinge point. Papain cleaves a site very close to the globular headpieces; these then separate to form 2 subfragments, each known as an SF-1 (for subfragment 1). The remaining superhelical portion of the molecule is known as SF-2. The ATPase activity of the myosin is associated with the SF-1 units.
A thick filament is composed of approximately 400 myosin molecules, 200 arrayed on either side of the M line. These molecules are maintained in bundles by C protein (clamp protein), M line protein and the hydrophobic interactions of the myosin molecules themselves. The myosin molecules are most tightly packed in the regions represented by the LMM portion of the molecules.
At the trypsin hinge point the heavy meromyosin angles sharply outward from the main axis of the thick filament. This extension of the heavy meromyosin away from the main axis of the thick filament helps bring the headpiece into close proximity to the actin thin filaments lying between the thick filaments. The molecular event underlying muscle contraction is the regulated binding of the myosin headpieces to actin thin filaments, followed by rapid myosin conformational changes about its hinge points with the bound actin being translocated toward the M line.
back to the top
Organization of Actin Thin Filaments
Thin filaments are composed of many subunits of the globular protein G-actin (42 kD) and several accessory proteins. In thin filaments, G-Actin is polymerized into long fibrous arrays known as F-actin. A pair of linear F-actin arrays is helically wound to form the backbone structure of 1 complete thin filament.
Each G-actin subunit has 1 ADP/ATP binding site, presumed to be involved in polymerizing the thin filament. Once polymerized, the actin is capped and the thin filament stabilized by a protein known as b-actinin. In addition to its nucleotide binding site, each G-actin molecule contains a high-affinity myosin head--binding site. In skeletal and cardiac muscle, accessory proteins of the thin filament (described below) physically regulate the availability of this site for binding myosin. Thus, the accessory proteins control contractile events.
Accessory Proteins of the Thin Filaments
The main thin filament accessory proteins are tropomyosin and troponin.Tropomyosin is a long, rod-like, a,b helically-interwound heterodimer that spans a length of 7 G-actin residues. A pair of tropomyosin molecules is associated with every 7 pairs of G-actin residues along a thin filament, 1 tropomyosin molecule in each of the grooves of the F-actin helix. In relaxed muscle, each tropomyosin molecule covers the myosin binding sites of 7 G-actin residues, preventing interaction between actin and myosin and thus maintaining the relaxed state. The onset of contractile activity involves activating troponin, the second accessory protein of thin filaments. Troponin is a heterotrimer attached to one end of each tropomyosin molecule and to actin, physically linking tropomyosin to actin.
Conformational changes in the bridging molecule, troponin, are responsible for moving tropomyosin on and off myosin binding sites of actin and thus regulating muscle contraction. One of the troponin subunits, troponin-C (Tn-C), is a calmodulin-like calcium-binding protein. When Tn-C binds calcium, the whole troponin molecule undergoes the conformational change that moves the attached tropomyosin away from the myosin binding sites on actin. This event permits nearby myosin heads to interact with myosin binding sites, and contractile activity ensues.
Events on the thin filament can be summarized as follows: Prior to the appearance of free calcium in the sarcoplasm, tropomyosin covers the myosin binding sites on actin. The appearance of calcium in the sarcoplasm leads to calcium binding on Tn-C. The resulting conformational changes in troponin move the attached tropomyosin molecule more deeply into the helix groove of F-actin, uncovering the myosin binding sites on G-actin subunits. The exposed sites are then available to interact with myosin headpieces. Removing calcium from the sarcoplasm restores the original conformational states of troponin and tropomyosin, preventing interaction between actin and myosin and leading to the relaxed state.
back to the top
Myosin and the Power Stroke of Contraction
In a rested, non-contracting muscle, myosin binding sites on actin are obscured and myosin exists a in high-energy conformational state (M*), poised to carry out a contractile cycle. The energy of ATP hydrolysis is used to drive myosin from a low-energy conformational state (M) to the high-energy state, as illustrated in Equation 1.
(M-ATP) <-----> (M*-ADP-Pi) Eqn. 1
When cytosolic calcium increases and myosin binding sites on actin become available, an actomyosin complex is formed, followed by the sequential dissociation of Pi and ADP with conversion of myosin to its low-energy conformational state. These events are accompanied by simultaneous translocation of the attached thin filament toward the M line of the sarcomere. The latter events, summarized in Equations 2 and 3 comprise the power stroke of the contractile cycle. Note that the energy of the power stroke is derived from ATP, via ATP-driven conversion of a low-energy myosin conformational state to a high-energy conformational state. A useful analogy is that ATP cocks the myosin trigger and the formation of an actomyosin complex pulls the trigger, releasing the energy stored in cocking the trigger.
(M*-ADP-Pi) + A <----> (M*-ADP-A) + Pi Eqn. 2
(M*-ADP-A) <-----> (M-A) + ADP Eqn. 3
At the end of the power stroke the actomyosin complex is remains intact until ATP becomes available. ATP binding to myosin is a very exergonic reaction, with the result that ATP displaces actin from the myosin head as indicated by Equation 4. Thus it is often said that ATP is required for muscle relaxation. It is important to note that in relaxed muscle, myosin is in its high-energy conformational state. Note that in Equation 4 the final product (M-ATP) is also the first reactant shown in Equation 1, completing the reactions of the contractile cycle.
(MA) + ATP <------> (M-ATP) + A Eqn. 4
A diagrammatic illustration of the reactions described in equations 1 through 4, as they occur in muscle, is shown below.
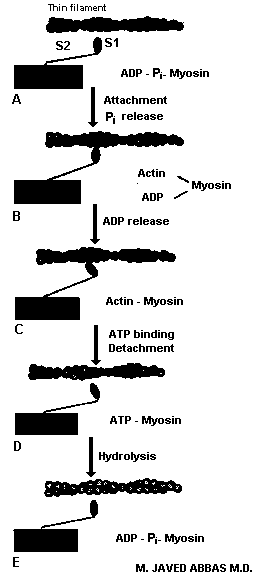 |
The process of reactions that occur leading to muscle contraction. A contractile cycle begins with myosin in a high energy conformation depicted by A. The power stroke begins with actin binding myosin in the A conformation and ends with formation of a low energy actomyosin complex, depicted in C. The complex is broken by ATP binding (step C to D) and the high energy conformation of myosin regenerated by ATP hydrolysis (D to E). Notice that the conformation of myosin in A and E is identical.
|
back to the top
Regulation of Sarcoplasmic Calcium
Events that stimulate muscle activity by raising sarcoplasmic calcium begin with neural excitation at neuromuscular junctions. Excitation induces local depolarization of the sarcolemma, which spreads to the associated T tubule system and deep into the interior of the myofiber. T tubule depolarization spreads to the sarcoplasmic reticulum (SR), with the effect of opening voltage-gated calcium channels in the SR membranes. This is followed by massive, rapid movement of cisternal calcium into the sarcoplasm close to nearby myofibrils. The appearance of calcium very close to the Tn-C subunit of troponin results in the production of multiple myosin power strokes, as long as the available calcium concentration remains greater than about 1 to 5 micromolar.
back to the top
Muscle Relaxation
Normally, cessation of contractile activity and a state of relaxation follow electrical quiescence at the myoneural junction. The sarcoplasmic membrane returns to its resting electrical potential (about 60 mV more positive outside), as does the entire T tubule system and the SR membrane. Subsequently, sarcoplasmic calcium is pumped back into the SR cisternae by an extremely active ATP- driven calcium pump, which comprises one of the main proteins of the SR membrane. For each ATP hydrolyzed, 2 calcium ions are moved out of the sarcoplasm, with sarcoplasmic calcium ultimately falling below 0.1 micromolar, or 50- to 100-fold lower than the KD for calcium binding to Tn-C. The cisternal surface of the SR membrane also contains large quantities of a glycoprotein known as calsequestrin. Calsequestrin avidly binds calcium, decreasing its concentration in the cisternae, and thus favoring calcium accumulation. A final repository of sarcoplasmic calcium is the mitochondrial matrix. Mitochondria have a remarkably active calcium pump, driven by the electron transport--generated chemiosmotic potential. Under aerobic conditions this pump uses the energy of electron transport to sequester calcium in the mitochondrial matrix, in preference to the synthesis of ATP.
back to the top
Tetany and Rigor Mortis
Tetany, a condition of hypercontracted muscle that sometimes follows a prolonged period of repetitive, summed muscle stimulation, is caused by the depletion of ATP and other high-energy phosphates that help maintain normal ATP levels. The latter include other nucleoside triphosphates (NTPs), creatine phosphate (CP), and ADP, as illustrated in Equations 5, 6, and 7. The three reactions are carried out by nucleoside diphosphokinase, creatine kinase and adenylate kinase, respectively.
NTP + ATP -------> NDP + ATP Eqn. 5
CP + ADP ------> Creatine +ATP Eqn. 6
ADP + ADP -------> AMP + ATP Eqn. 7
Since tetanic stimulation raises sarcoplasmic calcium and depletes ATP, the end result is a highly contracted muscle with calcium bound to Tn-C and no ATP available to resequester calcium into the cisternae of the SR, nor to break actomyosin cross-bridges. Under these conditions, mitochondria will preferentially pump calcium into the mitochondrial matrix, ultimately removing calcium bound to Tn-C, obscuring myosin binding sites on thin filaments, and, allowing the muscle to assume a flaccid state. However, the absence of ATP results in myosin remaining in its low-energy conformational state, with the result that new cycles of muscle stimulation will result in only limited ability of the muscle to generate contractile activity. Muscles in this physiological state are said to be fatigued.
In death, all reactions tend toward equilibrium. Among the first of these processes is that of ion equilibration across all compartments of the body as ion pumps loose their energy supplies. In the case of muscle, this results in cisternal and extracellular calcium leaking into the sarcoplasm, raising calcium concentrations to high levels. The calcium induces conformational changes in the troponin-tropomyosin complex, exposing myosin binding sites on thin filaments. The resulting uncontrolled contractile activity hastens the total exhaustion of ATP supplies and ends with all or nearly all myosin molecules in cross-linked actomyosin complexes. The rigid state of muscles that develops shortly after death is due to this highly cross-linked state of thin and thick filaments and is known as rigor mortis.
back to the top
Smooth Muscle
While the sliding filament model adequately describes the basic mechanism of contraction in all muscle types, there are significant differences between skeletal and smooth muscle. An appreciation of these differences stems from the observation that although smooth muscle lacks troponin, its contractile activity is still regulated by cytoplasmic calcium levels. This was explained when it was discovered that a Ca2+/calmodulin (CaCM) binding protein known as caldesmon was involved in regulating the movement of smooth-muscle tropomyosin on and off the myosin binding sites of thin filaments. The emerging picture, is that the elevation of cytosolic calcium raises the level of CaCM which, in turn, binds caldesmon, removing it from its site on thin filaments. Concurrently, tropomyosin is observed to change its location in the helical grooves of F-actin, and actomyosin ATPase activity is stimulated. When calcium is depleted the CaCM complex dissociates; caldesmon is released from its complex with calmodulin and reassociates with thin filaments. Actomyosin ATPase activity is correspondingly inhibited. In essence, caldesmon replaces troponin as a calcium (CaCM)-dependent regulator of tropomyosin's location on thin filaments.
A second major difference between smooth and striated muscle is that smooth muscle contains a unique myosin light-chain subunit known as p-light chain. P-light chain exists in phosphorylated and non-phosphorylated states, with its phosphorylation being determined by the activity of another CaCM-dependent protein known as myosin light chain kinase (MLCK). In the absence of CaCM smooth muscles are quiescent, the kinase is inactive, and p-light chains are not phosphorylated. Elevated CaCM levels induce MLCK activity, leading to phosphorylation of p-light chains and the onset of contraction. Both phosphorylation of p-light chains and the removal of caldesmon from thin filaments are required for smooth muscle contraction. This pathway of enzyme regulation via phosphorylation in smooth muscle is shown below.
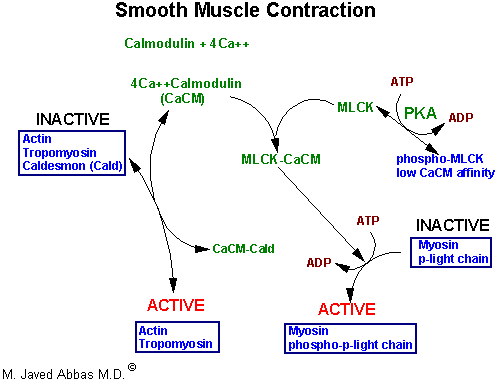 |
Flow diagram of enzyme activities during contraction in smooth muscle. Elevation of smooth muscle calcium to about 10-5M induces formation of Ca2+Calmodulin (CaCM) complexes which activate thin filaments by binding caldesmon (Cald) and freeing myosin binding sites on thin filaments. CaCM also binds and activates myosin light chain kinase (MLCK). Active MLCK phosphorylates myosin p-light chain activating the actomyosin ATPase activity of myosin headpieces. Epinephrine binding to b-adrenergic receptors raises cAMP, activates cAMP-dependent protein kinase (PKA), which reduces the affinity of MLCK for CaCM and modulates the strength of contractions generated by elevated cytosolic calcium.
|
back to the top
Red Oxidative and White Glycolytic Muscles
In addition to the phosphoryl transfers described by above in equations 5, 6, and 7, muscle ATP is also generated by glycolysis and oxidative phosphorylation. Muscles that depend predominantly on oxidative phosphorylation for ATP require abundant oxygen. To ensure its availability, these muscles store appreciable oxygen as oxymyoglobin. Oxidative, myoglobin-containing muscles are red in color because of their high myoglobin content. Glycolytic muscles lack appreciable myoglobin and appear white. These muscle generally contain abundant stores of glycogen and generate most of their ATP from glycolytic reactions. A major functional difference between red and white muscle cells is that white fibers generate ATP by a short reaction pathway between substrates (eg, glucose) and the appearance of ATP, whereas in red muscle the pathway from substrate (again, glucose) to ATP is comprised of many more reaction steps (eg, glycolysis plus TCA cycle plus electron transport) and is a correspondingly longer process. Consequently, fast-acting skeletal muscles are composed of dominantly glycolytic white fibers while slow-acting muscles such as those that maintain tone are generally red and oxidative.
back to the top
Back to Topics<<<<
This article has been modified by Dr. M. Javed Abbas. If you have any comments please do not hesitate to sign my Guest Book.
20:29 19/12/2002
|